Early-universe soup
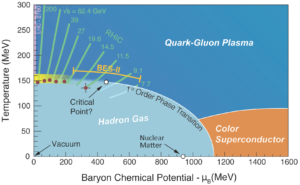
An experimental and theoretical exploration of the quantum chromodynamics (QCD) phase diagram. (Click to enlarge.) The matter produced in collisions at the highest energies and the smallest baryon chemical potentials can change from quark-gluon plasma (QGP) to a hadron gas through a smooth crossover. But lower energy collisions can access higher baryon chemical potentials where a first-order phase transition line is thought to exist. The reach of the future DOE Basic Energy Sciences program at RHIC is shown, as are the trajectories on the phase diagram followed by the cooling droplets of QGP produced in collisions with varying energy. The present reach of lattice QCD calculations is illustrated by the yellow band. (Illustration: Swagato Mukherjee, Brookhaven National Laboratory.)
At the dawn of the universe – just after the Big Bang – all matter was in the form of a hot-flowing soup called quark-gluon plasma, or QGP. Though a few ambitious, atom-smashing experiments have produced transient samples of this extreme phase of matter, researchers still have much to learn about its fundamental behavior.
Experimental physicists have tried to produce quark-gluon plasma since the 1980s and first reported observing it in 2000. Over the past 45 years, theorists have outlined the equations that govern QGP and many have combined theory and experiment to describe it.
Large-scale computations have been critical to the theoretical study of QGP’s novel characteristics. As part of a theoretical effort funded by the Department of Energy, Brookhaven National Laboratory’s Swagato Mukherjee and his colleagues are using an allotment of 167 million processor hours from the ASCR Leadership Computing Challenge (ALCC) to better understand QGP. Their findings will help physicists plan the next wave of experiments. “Neither theory nor experiment can do this alone,” Mukherjee says.
At the heart of every atom lies the nucleus, a super-tight ball of subatomic protons and neutrons. Those particles are made of even smaller parts, including quarks, which comprise just one thousandth of the mass. Gluons, the adhesive particles that hold quarks together, carry the strong interaction, a fundamental physical force that binds the atomic nucleus and generates the other 99.9 percent of all matter’s mass.
But at temperature extremes 70,000 times hotter than the center of the sun, even tightly packed quarks and gluons begin to flow. The transition to the flowing state is much like phase changes in matter such as water. Water exists as liquid, steam or ice, based on how much heat and pressure are applied. Scientists long ago carefully mapped the underlying conditions and boundaries between water’s different forms as a phase diagram, information that’s been critical for understanding water’s behavior. If researchers can understand how changes in temperature and density affect QGP, physicists can create a similar roadmap documenting conditions that form it.
Because of the extreme conditions required for QGP creation, the only way to observe it on Earth is to bombard matter with high-energy particles at either the Relativistic Heavy Ion Collider (RHIC) at Brookhaven or the Large Hadron Collider at CERN in Switzerland. Fast-moving nuclei of lead and gold collide at high energy, briefly producing the plasma-soup researchers can study.
Experiments aren’t the only way to study QGP’s properties. Physicists have worked out the theory of how quarks and gluons interact, known as quantum chromodynamics, or QCD. However, the complexity of these interactions, with billions of variables, requires sophisticated parallel computing resources to solve, Mukherjee says.
Using their ALCC allotment, Mukherjee and his colleagues have concentrated on a version of this theory, lattice QCD, to computationally study the plasma on Titan, a Cray XK7 at Oak Ridge National Laboratory. The calculations line up quarks at the intersection points on a grid, with gluons positioned on each of the crossbars between them. Initially, the researchers omitted the density component and solely calculated how increasing heat eventually produces the flowing QGP. Now they’ll need to consider the density component as well. With their ongoing ALCC allotment, they’re simulating how increasing density changes the phase diagram and eventually the plasma’s behavior.
These types of computations will be critical for future experiments at the big colliders. In 2019 and 2020, DOE will support a large collaborative effort, the Beam Energy Scan II at RHIC, to observe the full phase diagram of quark-gluon plasma, including the density component, Mukherjee says, an effort that will cost hundreds of millions of dollars. The calculations Mukherjee and his colleagues perform will provide information that helps the experimental physicists plan those experiments. The calculations will provide temperature benchmarks – a range needed to generate QGP.
In large particle accelerators, researchers can’t control the temperature or density, only the energy of the atomic collisions, Mukherjee says. So calculations will help researchers translate that collision energy into the heat and density parameters they need to observe the full range of changes in the phase diagram of quark-gluon plasma.
Ultimately, the exercise is about fundamental discovery and collaboration between theorists and experimentalists to discover the quark-gluon soup recipe. Mukherjee is part of a larger Brookhaven theoretical team, the Nuclear Physics Lattice Gauge Theory group led by Fritjof Karsch. This work is an integral part of the BEST collaboration – for Beam Energy Scan Theory – a DOE-funded, multi-institutional Topical Collaboration in Nuclear Theory, looking at the phases and properties of hot-dense QCD matter. Mukherjee’s research is supported by DOE Office of Science’s Nuclear Physics program.
About the Author
Sarah Webb is science media editor at the Krell Institute. She’s managing editor of DEIXIS: The DOE CSGF Annual and producer-host of the podcast Science in Parallel. She holds a Ph.D. in chemistry, a bachelor’s degree in German and completed a Fulbright fellowship doing organic chemistry research in Germany.