Forged in a Firestorm
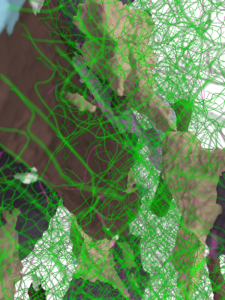
A dense network of lattice defects develops in a flowing tantalum crystal, but it maintains an ordered structure that preserves its strength and stiffness. (Visualization: Alexander Stukowski, Technische Universität Darmstadt. )
A medieval blacksmith making a sword knew that pounding on iron altered its shape. At the atomic level, that same mechanism – crystal plasticity – has remained somewhat speculative, but materials scientist Vasily Bulatov is changing that.
Long after the Iron Age, scientists concluded that atoms sliding by each other cause a material’s shape to change. Still, “it’s impossible to observe and quantify exactly how the atoms do it,” says Bulatov, a senior scientist at the Department of Energy’s (DOE’s) Lawrence Livermore National Laboratory (LLNL). Thus, scientists can’t watch the atomic movement deep in the bulk metal, and “it has been too expensive to model on a computer, although generally it was understood how to do these simulations. It’s kind of funny that no one doubts that this connection exists, but no one has been able to actually compute or predict that.”
After 25 years of interest in crystal plasticity, Bulatov hopes to use first principles – the basic rules of physics – to solve two key controversies in this field. He already described some of the tools the job requires: In 2017, he and LLNL colleagues Tomas Oppelstrup and Luis Zepeda-Ruiz , with Alexander Stukowski of Germany’s Technische Universität Darmstadt, reported in Nature that they could simulate crystal plasticity in the metal tantalum.
“Physicists have been fighting over 80 years about why crystals deform plastically,” Bulatov says. “One controversy is why a crystal hardens if you pull on it, and the more you pull the stronger it becomes until it breaks.” This so-called strain hardening is a three-stage process: a little, then a lot, then a little. This property is found in many crystals, but scientists still debate what causes it.
In 2018, Bulatov and his colleagues used immense simulations of crystals – comprised of 250 million to 4 billion atoms – in an effort to replicate this behavior. They spent half a year working on tantalum, which didn’t show three-stage hardening because the simulations model only high rates of straining (low-rate simulations are too computationally expensive) and the element’s high lattice resistance impedes the process.
The team turned to aluminum, producing useful results that revealed the mechanism behind the three-stage process. “It’s now in our pocket,” Bulatov says, and the team is preparing a paper.
The other longstanding controversy involves dislocation patterns. Since the 1930s, some scientists have suggested that only a small fraction of a crystal’s atoms slide at any one time during plasticity. Under this theory, the movement of active atoms arranged in a continuous line – a dislocation – causes a crystal to deform. It took another 25 years before transmission electron microscopy (TEM) provided enough resolution to see that dislocations actually exist.
“Once we learned to see the dislocation lines, it became immediately obvious they do not make a spaghetti soup,” Bulatov says. “Most of the crystal remains free of these lines, and they cluster and form patterns, like braids, walls or cells.” Which patterns form depends on the material and how it is strained.
Though everyone now agrees that dislocation patterns exist, not all believe they play a role in crystal plasticity. Because TEM needs thin sections of material to image dislocations, scientists can’t strain the crystal under it to form patterns.
That leaves questions, Bulatov says. “Do these patterns form during straining or after you take the specimen from the straining machine and put it on a shelf for weeks? We’re hoping this year that, with the help of new simulations, we can shed light on such questions.”
With TEM, scientists look closer and closer at the dislocation patterns. Some see cell-like structures, thick-walled lines with nothing inside; others see fractal-like patterns that are similar on all scales. “The very same TEM images have been used, depending on the prevailing views of the time, to justify cell-like patterns and fractal-like patterns,” Bulatov says.
He is working with that same Nature-author team to get the most from an INCITE allocation of 17 million node-hours at the Argonne Leadership Computing Facility, a DOE Office of Science user facility, on Mira, its IBM Blue Gene/Q supercomputer. The team will use a molecular dynamics method, which Bulatov describes as “something we’ve been working on for 60 years or more, and it’s mostly unchanged – nothing new there.”
What is new is how the team will handle the results. “If you just look at atoms and how they move, it’s overwhelming and totally uninformative,” Bulatov says. “You need to see inside, see what’s going on there.” So from a huge amount of data, the team needs a way to pull out the useful parts. “It’s our methods of analysis and data reduction that make our project special. We’re fortunate to have (Technische Universität Darmstadt’s) Alex Stukowski, who develops beautiful algorithms to extract information from humongous simulations.”
In this work, Bulatov’s team plans to focus on the geometric patterns. “We have a hunch that they are both cells and fractals,” he says.
Beyond addressing the questions of strain hardening and dislocation patterns, Bulatov sees an even bigger reason to perform these simulations. “In the larger view of things, we’d like to demonstrate that it’s possible to tackle crystal plasticity at the most fundamental level from first principles,” he says, something dismissed as impossible before the Nature paper.
Now that he’s shown that crystals can be studied atom-by-atom – all derived from molecular-dynamic simulations – he wants to go even further. “We’d like to show that you can do even more with this and extract useful scientific insights that otherwise would remain hidden forever.”
These simulations require the world’s fastest supercomputers. As machines get more powerful, Bulatov sees even more opportunities ahead. “In five to seven years, we will be able to do something absolutely amazing.” Maybe even sooner, he hopes to develop a virtual lab where scientists can work with crystal plasticity that is completely predicted from first principles.
About the Author
Mike May has worked as a full-time freelancer since 1998, covering topics ranging from biotech and drug discovery to information technology and optics. Before that, he worked for seven years as an associate editor at American Scientist. He earned an M.S. in biological engineering from the University of Connecticut and a Ph.D. in neurobiology and behavior from Cornell University.
You must be logged in to post a comment.