Enhancing enzymes
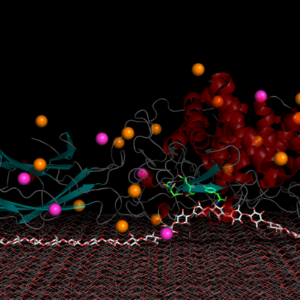
A model of cellulase acting on a cellulose substrate. The enzyme latches onto a cellulose chain, and the green colored residues on the enzyme help to release the smaller sugar units. Other enzymes then convert these simple sugars into bioethanol. (Image: Oak Ridge National Laboratory.)
Enzymes are the workhorses of almost all processes in living systems. They can convert light into chemical energy and remove carbon dioxide from the atmosphere and oceans. Because of these natural superpowers, Pratul Agarwal of the Department of Energy’s Oak Ridge National Laboratory (ORNL) would like to harness enzymes’ catalytic capabilities to improve biofuels production and carbon capture.
A computational biochemist and biophysicist, Agarwal has long been driven to understand the details of enzymes and how they work. While earning his Ph.D. at Pennsylvania State University, he worked with renowned computational chemist Sharon Hammes-Schiffer (now with the University of Illinois at Urbana-Champaign) to model how enzymes optimize chemical reactions. At that time, much of the biology community was skeptical about these computational tools, Agarwal says. “The quality of models was not great,” he concedes. “If you made a prediction, it wasn’t actually very good.”
Then, in 2002, he started work as a research scientist at ORNL, where one of the first supercomputers was under construction. The position let Agarwal meld his interest in high-performance computing with his desire to engineer biological catalysts. Over the past 15 years he’s focused on building high-quality predictive models of enzymes. These computational tools let his team reproduce important data from experiments and then predict how protein sequence variations and other modifications affect enzymes’ function. “So we understand much better how enzymes work and what makes them work faster,” Agarwal says.
The computational challenges are daunting. In a typical model, Agarwal must account for detailed movements and processes in enzymes’ active sites. But a high-quality model can’t focus only on those small-scale details, so he also has to zoom out and examine movements and forces across the whole enzyme. This means looking at tens of thousands of atoms both in the enzyme and in the surrounding water. Timescales are also challenging: Once enzymes are locked and loaded, the chemical process can occur within a few femtoseconds, but the overall catalytic process can last more than a billion times longer. Agarwal is interested in the full catalytic cycle.
Laboratory biochemists originally went in with the idea that a portion of the enzyme acts as a lock, whereas the substrate – the molecule it acts on – serves as a key. Using a variety of strategies, they make either planned or random chemical changes to the enzyme’s basic sequence. Then they study whether those changes slow an enzyme down, speed it up or inactivate it. This divide-and-conquer approach to enzymes has helped explain a lot about how these proteins work, Agarwal says. But it doesn’t tell the whole story.
Computational models can speed up some of this experimental work, but they also can get an overall picture of how an enzyme works that is difficult to observe with experiments. Enzymes are much faster and larger than synthetic catalysts, but biochemists haven’t explained why these structures must be so big. Biological systems also must be efficient, which suggests that the thousands of atoms in these large structures are not just idle spectators but direct participants.
Agarwal’s models offer a unique tool for studying the overall dynamics – the movements in all parts of the enzyme, even those that are not directly interacting with the substrate. “Motion is energy,” he says, noting that once researchers learn where the important motions are, they can boost enzymes’ efficiency. From these studies, Agarwal has learned that roughly 10 percent of the atoms in the enzyme and in the surrounding water are involved in the energy flow that drives chemical reactions forward.
So far, Agarwal and various collaborators have looked at 15 different enzymes and modeled their underlying biophysics. They’ve also used these ideas to modify and accelerate enzymes. In the Journal of Physical Chemistry Letters in 2011, they added a light-activated molecular switch to an enzyme, which changed the underlying protein structure. Laboratory tests confirmed that the new protein was more than 30 times faster than the original enzyme.
That fundamental understanding of enzymes could prove useful for addressing two important renewable energy goals: harnessing energy from cellulose-based biofuels and capturing greenhouse gas carbon.
Many researchers have considered cellulase – the enzyme that breaks apart cellulose, a structural component of all plants – to produce fermentable sugars. Part of the problem is separating the cellulose from a plant’s other polymers, such as lignin. But even with clean cellulose, naturally occurring cellulases are not efficient enzymes.
Cellulases are found in bacteria or fungi that are surrounded by ample cellulose in such places as tree bark. Such small organisms only need a tiny amount of fermentable sugars to survive, so the enzyme doesn’t need to be fast. But for bioethanol production, researchers need a much speedier, cost-effective enzyme. So Agarwal’s models aim to eke out efficiencies.
Through computation, he and colleagues can test scenarios, saving time and limiting costly experiments. But it also allows them to examine an enzyme’s complicated inner workings – for instance, determining exactly where cellulose degradation stalls. Their initial simulations suggest that a speed bump occurs as the enzyme moves down the long chain of sugars that comprise the polymer. They hope to learn how the enzyme acquires energy from its environment and exactly how it harnesses that energy to cleave the cellulose’s chemical bonds. That information could lead to methods that remove kinks from this process.
As for carbon capture, computation can help there, too. Agarwal focuses on RuBisCO (short for ribulose-1,5-bisphosphate carboxylase/oxygenase), the first enzyme in a series of catalysts plants and algae use to remove carbon dioxide, which is ubiquitous in their surroundings. Nature also doesn’t push RuBisCO and other enzymes in this pathway to work quickly. Agarwal would like to speed things up. To do that, he asks different questions. Exactly how does this enzyme grab a molecule of carbon dioxide? And what bottlenecks in this multi-enzyme process slow down carbon capture? The team is still working on a solid predictive model of this enzyme. If successful, it could point toward designing algae or other cells that could capture carbon on demand.
So far, Agarwal’s computational work has been funded through various DOE programs, including through the INCITE (Innovative and Novel Computational Impact on Theory and Experiment) and ALCC – ASCR (Advanced Scientific Computing Research) Leadership Computing Challenge – programs, and ORNL internal funding. He seeks support for further computational work and vital experimental collaborations. The work remains ongoing, he says, “a labor of love.”
About the Author
Sarah Webb is science media editor at the Krell Institute. She’s managing editor of DEIXIS: The DOE CSGF Annual and producer-host of the podcast Science in Parallel. She holds a Ph.D. in chemistry, a bachelor’s degree in German and completed a Fulbright fellowship doing organic chemistry research in Germany.